All published articles of this journal are available on ScienceDirect.
Water Vapor Uptake and Microwave Heating of Magnetite / Aluminum Fumarate Composites
Abstract
Aim
This study aims to obtain new desiccant materials based on magnetic nanoparticles and metal-organic framework (MOF) that are regenerable by microwave heating.
Background
Among the nanoparticles/MOF composites, the magnetic ones are especially interesting as sorbents with stimuli-triggered release of adsorbate and separation/concentration in an external magnetic field.
Methods
The composites were obtained by adding pre-synthesized magnetite nanoparticles to the solution of fumaric acid used to synthesize the porous aluminum fumarate (Al-fum) matrix. The mass fraction of Fe3O4 in the composites was evaluated by an o-phenanthroline-based assay, and their structure was characterized by XRD and FTIR. The magnetic properties and humidity-dependent water vapor uptake were measured.
Results
The broad X-ray diffraction signals of magnetite, decreased Curie temperature, and increased water uptake by the composites with a low magnetite content reflect the escalation of microdefects at the interphase boundary. Higher temperatures and pressures were reached in a shorter period of time upon the microwave heating of water-enriched powders and aqueous dispersions of Fe3O4/Al-fum composites as compared with pure water.
Conclusion
The absorption of microwave radiation by Al-fum saturated with water is improved to a degree that allows one to overcome the decrease in dielectric losses and tangent of pure water with increasing temperature. The presence of magnetite in the amount of 0.04 g g-1 or more accelerates the heating of aqueous dispersions of the composites to the same extent as pure magnetite nanoparticles.
1. INTRODUCTION
Metal-organic frameworks (MOFs) are of great interest due to their periodic 3D structure with interconnecting system of voids accessible for selective filling with small molecules and related extraordinary porosity that manifests itself in a high specific surface area and adsorbate uptake. The possibility of synthesis from block compounds and flexible use of aqueous and non-aqueous solvents, temperature, and modulators allow one to obtain MOFs with precisely specified structure and physicochemical properties, often as a layer on the template surface [1-4]. By embedding metal, metal oxide, ceramic, and polymeric nanoparticles into the bulk porous matrix or surrounding them with a shell of MOF, new composites with a unique set of properties are obtained. As expected, they consolidate tailorable porosity and adsorption selectivity of MOF with the catalytic activity of nanoparticles into new functional material with enhanced performance. Possible applications of such composites cover catalysis, adsorption, storage, and separation of gases, plasmonic sensing, sequestration of pollutants, molecular drug delivery, etc [5-9].
Among the nanoparticles/MOF composites, the magnetic ones are especially interesting since they can be recollected by applying an external magnetic field to separate/concentrate the sorbent with inorganic pollutants or target organic compounds [7, 10, 11]. Furthermore, they become sensitive to alternating magnetic fields or microwaves to stimuli-trigger the release of adsorbate molecules (water, CO2, H2, therapeutics) [6, 12-16].
The physico-chemical effects related to the absorption of microwave radiation (heating, water desorption, catalytic performance, etc.) have been reported for a limited number of pure MOFs and MOF–based composites [9, 13-17]. As broadband microwave-absorbing materials, they are inferior to MOF-derived carbon-based composites. The latter obtained by pyrolysis of MOFs or metal-enriched MOFs in air or inert atmosphere keep high specific surface area and porosity inherent in MOF and demonstrate effective electromagnetic wave absorption in the range of 3.5 to 8 GHz as a few millimeter coating, while relatively few MOF–derived coatings absorb below 3 GHz [18].
We report here on the synthesis of magnetic nanoparticles/MOF composites with different magnetite to aluminum fumarate (Al-fum) ratios, in which magnetite nanoparticles are incorporated into a porous MOF matrix, the study of their composition and structure, including the mutual influence of the two phases, as well as water vapor uptake by the composites and influence of the water saturation on the 2.45 GHz microwave heating.
Magnetic iron oxides are thermally stable in air up to temperatures of 750 K, which ensures their wide application in many industries. Fe3O4 nanoparticles have unique characteristics: chemical stability, low cost and biocompatibility, proven by their applications in such fields as cell labeling and drug delivery, as contrast agents for MRI or magnetic heating mediators [19, 20]. Aluminum fumarate MOF is considered as a promising environmentally friendly adsorbent for a high variety of applications with exceptionally high hydrothermal stability [21-24]. Preliminary evaluation showed the good reproducibility of water uptake by selected Fe3O4/Al-fum composites under humid conditions [25]. The magnetite nanoparticles included in the MOF matrix are expected to improve the water absorption properties of the composites and enhance their sensitivity to microwave heating.
2. MATERIALS AND METHODS
2.1. Materials
Al2(SO4)3·18H2O (97%, Sigma-Aldrich), fumaric acid (98%, Sigma-Aldrich), FeCl3·6H2O (98%, Sigma-Aldrich), FeSO4·7H2O (99%, Sigma-Aldrich), NaOH (99%, Sigma-Aldrich), toluene (Sigma) were used as received. Distilled water was obtained one day prior to the experiments using a laboratory distiller.
2.2. Synthesis of Magnetite
Magnetite was synthesized from аn aqueous solution of iron salts [26]. In a typical experiment, 100 mL of a 0.125 M FeCl3 solution and 50 mL оf a 0.069 M FeSO4 solution were combined and stirred for 3 min, then the mixture was added to a 0.338 M sodium hydroxide solution (200 mL), further stirred for half an hour under constantly monitored pH of 11. The particles were washed by magnetic decantation to neutral pH and brought to a ca. 21.5 g L-1 concentration in terms of solid matter by adding distilled water. The hydrodynamic diameter of the obtained magnetite nanoparticles measured by dynamic light scattering is approximately 30 nm.
2.3. Synthesis of Fe3O4/Al-fum Composites
In order to incorporate the freshly synthesized magnetite nanoparticles into porous aluminum fumarate matrix the synthetic method was modified [3]. А predetermined volume of the magnetite dispersion was taken to achieve a given theoretical ratio of magnetite to Al-fum, namely 1:х, where x = 1, 5, 10, 20, 50, in the composites and admixed to a solution obtained from fumaric acid (1.05 M, 200 mL) and sodium hydroxide (3.94 M, 160 mL). The resulting mixture was added to 300 mL of a 0.35 M aluminum sulfate solution for 10 min with constant stirring. The dark to light brown precipitates were separated by vacuum filtration through a paper filter. They were dried at 100°C in the air for 24 h followed by activation in a vacuum oven at 130°C for 24 h [27]. The composites were fine ground in a mortar.
2.4. Characterization of Fe3O4/Al-fum Composites
The mass fraction of magnetite in the composites (ω) after their dissolution in НCl was determined by an o-phenanthroline-based assay for total iron [28] using a CM 2203 Solar spectrofluorimeter. FTIR spectra of the Fe3O4/Al-fum composites were recorded on a Tensor 27 Bruker spectrometer after pressing them into KBr tablets. A scanning electron microscope LEO 1420 was used to characterize the morphology of composites. X-ray diffraction analysis (XRD) was performed on an X-ray diffractometer DRON-3 using a Co Kα1 (α = 1.79021 Å) radiation source. The water uptake by the powders was measured using a MAX 50 Radwag moisture analyzer. The powder under investigation was placed into a cell with controlled relative humidity (RH) and temperature for 24 h, then quickly poured onto the analyzer weighing pan and heated at 80 °С until a constant mass was achieved.
The temperature dependence of specific magnetization (σ) was measured in the range of 77 – 900 K by the Faraday method in a magnetic field of 0.86 T. The error in magnetization measuring is ±0.005 emu g-1.
The interaction of the Fe3O4/Al-fum composites with microwave radiation was evaluated using a MonoWave 300 Anton Paar instrument operating at a frequency of 2.45 GHz. The composite under study was dispersed in either toluene (8.5 mg mL-1) or water (8.5 and 250 mg mL-1) and a 5.5 mL aliquot of the dispersion was irradiated at a 30 W power in a sealed microwave vial under constant steering. The irradiation of solvent-free composite powders (5.5±0.1 g) was carried out after drying them over KOH flakes. Otherwise, the composites were kept at a relative humidity of 90% in a sealed container for 12 h at 20±10C prior to microwave heating. The pressure (P) and temperatures of the wall (TIR) and internal content (TR) of a microwave vial were measured with a time interval of 1 s. The microwave heating was automatically stopped after reaching one of the preset upper limits (200 0C and 16 (30) bar for temperature and pressure, respectively) or manually interrupted after no perceptible increase in temperature occurred within 60 s.
The standard deviations of triply determined time of heating to 200 0C of saturated with water vapor powders of Al-fum (sample-to-sample) and a 0.06 g g-1composite (trial-to-trial) were ca. 42 and 28 s, respectively. For aqueous composite dispersions, good reproducibility in microwave heating experiments was also observed; the standard deviation in a series of three heating time measurements does not typically exceed 30 s.
3. RESULTS AND DISCUSSION
By admixing various amounts of Fe3O4 nanoparticles to a sodium fumarate solution before adding the latter to aluminum sulfate, the Fe3O4/Al-fum composites with the different ratios of Fe3O4 to Al-fum were prepared (Table 1). The mass fractions of magnetite in the composite particles were determined by an o-phenanthroline assay and also calculated as the ratio of specific magnetization of the composites to that of magnetite nanoparticles as prepared. The obtained values are in good agreement with each other. The experimental values of ω in the composites appear to be approximately 30% lower than the theoretically calculated magnetite fractions. However, the calculations did not take into account water that the Al-fum-based composites absorb at ambient temperature and relative humidity, leading to overestimated values of ω. Meanwhile, the water uptake by such a composite, as shown below, can reach 300 mg g-1.
The microphotograph of a Fe3O4/Al-fum composite powder in Fig. (1a) shows highly polydispersed aggregates with pronounced edges. They consist of small MOF crystals that are responsible for rough surface appearance, as can be seen at larger magnification Fig. (1b). Magnetite nanoparticles appear to be evenly distributed throughout the Al-fum matrix and embedded into the porous material; no bulk phase of iron oxide was visualized.
Composition 1:х |
ω, g g-1* | TСurie, К | σ, emu g-1 | ||
---|---|---|---|---|---|
Theory | Experiment | ||||
o-phen. assay | magn. | ||||
Fe3O4 | 1.00 | 0.982±0.035 | 1.00 | 805 | 23.0 |
1:1 | 0.50 | 0.341±0.007 | 0.326 | 800 | 7.5 |
1:5 | 0.17 | 0.114±0.015 | 0.109 | 790 | 2.5 |
1:10 | 0.09 | 0.057±0.003 | 0.057 | 760 | 1.3 |
1:20 | 0.05 | 0.039±0.009 | 0.035 | 750 | 0.8 |
1:50 | 0.02 | 0.013±0.004 | 0.011 | 730 | 0.3 |
Al-fum | 0.00 | 0.000±0.002 | - | - | - |
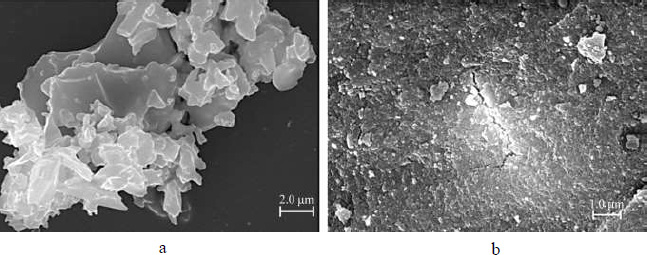
SEM of Fe3O4/Al-fum, ω = 0.34 g g-1 composite powder as prepared (a) and surface of a dried agglomerate of particles (b).
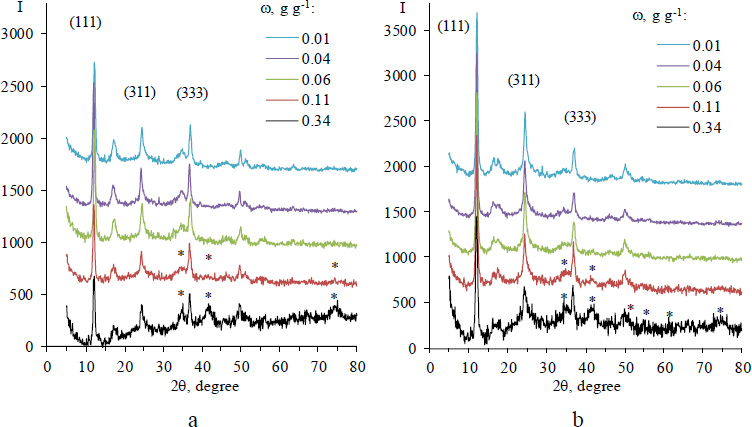
XRD spectra of Fe3O4/Al-Fum composites with different magnetite mass fractions before (a) and after (b) drying in a vacuum oven. The diffraction peaks that correspond to the reflection planes of aluminum fumarate are indexed. The diffraction reflexes of Fe3O4 are marked with an asterisk.
The X-ray diffraction spectra of Fe3O4/Al-fum composites are shown in Fig. (2). The positions and relative intensities of the peaks in the spectrum of Al-fum coincide with those reported for the MOF in [29, 30]. The spectrum of a composite with ω=0.34 g g-1 additionally contains broad peaks centered at 2θ of 35.5 and 42.0 that are typical for magnetite {311} and {400} reflection planes [31, 32]. In the spectra of the composites, some diffraction peaks of magnetite overlap with those of Al-fum and appear as broadening or shouldering of a main strong peak, such as one at 2θ ~35.5. The magnetite diffractions are barely noticeable in the XRD spectra of a composite with the magnetite mass fraction of 0.11 g g-1 and do not appear at lower ω despite other methods confirming the presence of magnetic phase.
It is worth mentioning that the intensity of X-ray reflections increases after drying the composites in a vacuum oven for 24 h. It is accompanied by some changes in the relative intensity of the peaks while their positions remain unchanged.
In contrast to the X-ray reflections of aluminum fumarate, the peaks of the iron oxide phase are greatly broadened and, regardless of ω are of low intensity. This well-known side effect is associated with the incompleteness of the processes of phase formation and crystallization of the spinel structure, the existence of defects in large quantities and disruption of the distribution of cations across the sublattices of the crystal structure, as well as the existence of a surface layer with a non-collinear arrangement of spins, which is caused by fluctuations of magnetic moments due to the significantly greater influence of thermal vibrations on ions, located on the surface of magnetite nanoparticles [33, 34].
The intense characteristic adsorption bands of Al-fum appear in the FTIR spectra of all Fe3O4/Al-fum composites Fig. (3). The detailed assignment of the peaks in the spectrum of Al-fum can be found elsewhere [31]. For pure magnetite nanoparticles, two closely situated merging peaks are observed at 592 and 632 cm-1, which can be attributed to the vibration of Fe-O bonds in the spinel structure [35]. Two wide asymmetric uninformative bands at 3460 and 1628 cm-1 in the magnetite spectrum are related to the presence of hydroxyl groups and adsorbed water molecules (O-H stretching and H-O-H bending vibrations respectively) [36]. Despite the presence of magnetite particles in all Fe3O4/Al-fum composites being confirmed by other methods (Table 1), only a moderate increase of absorption around 600 cm-1 and an asymmetric broadening of the band centered at 3487 cm-1 are noticeable in the spectrum of a 0.34 g g-1 composite because of overlapping with more intense absorption peaks of Al-fum.
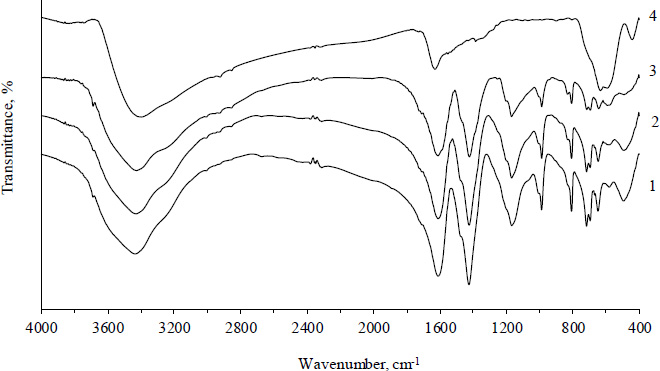
FTIR spectrum of Al-fum (1), Fe3O4/Al-fum composites (2-3, ω, g g-1: 2 - 0.11; 3 - 0.34), and Fe3O4 nanoparticles (4).
The surface layer fraction in fine magnetite particles is expectedly large; this results in decreasing their saturation magnetization as compared to bulk material [34, 37]. The saturation magnetization of the synthesized magnetite nanoparticles used for the preparation of Fe3O4/Al-fum composite is only 23.0 emu g-1, while the values for the composites depend on their composition. By comparing the magnetization values with that of pure magnetite, the mass fraction of the latter was independently evaluated (Table 1). The values of ω for the composites obtained by two independent methods align well with each other, thus, the preservation of the magnetic properties of nanoparticles incorporated in the porous MOF matrix is confirmed. This is in good agreement with magnetic properties of particulated magnetite reported previously [32].
The dependence of magnetic properties of the composites on temperature was investigated in order to evaluate their thermal stability. The curve of specific magnetization of pure prepared Fe3O4versus temperature shows that it behaves as a typical ferromagnetic Fig. (4). The Curie temperature (TCurie) for magnetite nanoparticles (Table 1) turned out to be lower than the value for bulk material (858 K) [38]. This fact is known for nanoparticles and is due to the existence of a surface layer with an imperfect crystalline and magnetic structure [39, 40]. We found that a decrease in the amount of magnetite in the composites led to a further slight decrease in the Curie temperature (Table 1). This may also indirectly reflect the influence of hydrophilic MOF matrix as magnetite surrounding the properties of the surface layer of the nanoparticles.
The water uptake by the freshly prepared Fe3O4/Al-fum composites with different mass fractions of magnetite is shown in Fig. (5). In an arid atmosphere (RH~10%), the water vapor absorption by the composites is low regardless of the concentration of magnetite. As the relative humidity rises to 70%, the positive effect of small amounts of fine magnetite particles becomes visible, apparently associated with an escalation of microdefects in the Al-fum lattice structure caused by the incorporated nanoparticles. The maximum water vapor uptake of 464 mg g-1 was found for ω around 0.04 g g-1. The increase is as high as 17% as compared with Al-fum matrix. With increasing the content of magnetite to 0.34 g g-1, the water uptake by the composite drops drastically below 250 mg g-1, thus approaching the value for finely ground magnetite powder (213 mg g-1). At the high relative humidity (RH=90%), freshly prepared pristine Al-fum and the composites with ω lower than 0.11 g g-1 show a high uptake of water vapor, and the adsorption reaches up to 600-650 mg g-1. The high water uptake value may be partially due to the condensation of vapor in the pores at high humidity [4]. A good reproducibility of water uptake by Al-fum and a 1:10 Fe3O4/Al-fum composite has been previously shown [25]. In six consecutive cycles of relative humidity alternation from 10% to 90% the coefficient of variation was less than 7%.
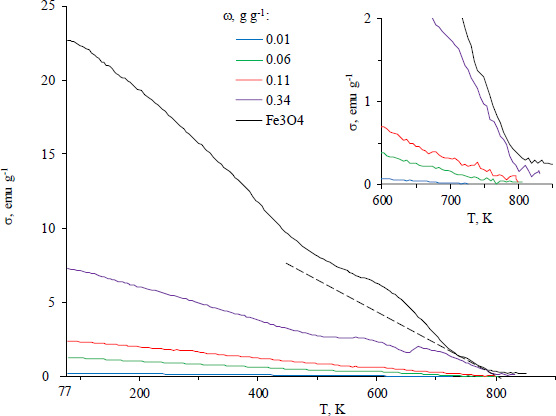
Dependence of specific magnetization of Fe3O4/Al-fum composites and Fe3O4 nanoparticles as prepared on temperature. The insert shows the region near the Curie temperatures of the composites.
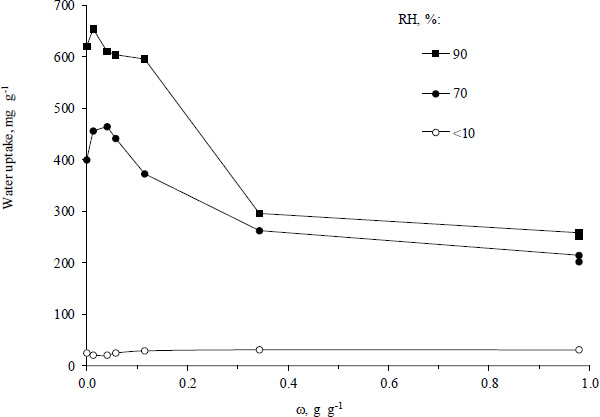
Water uptake by Fe3O4/Al-fum composites with different mass fractions of magnetite. 24±1°С.
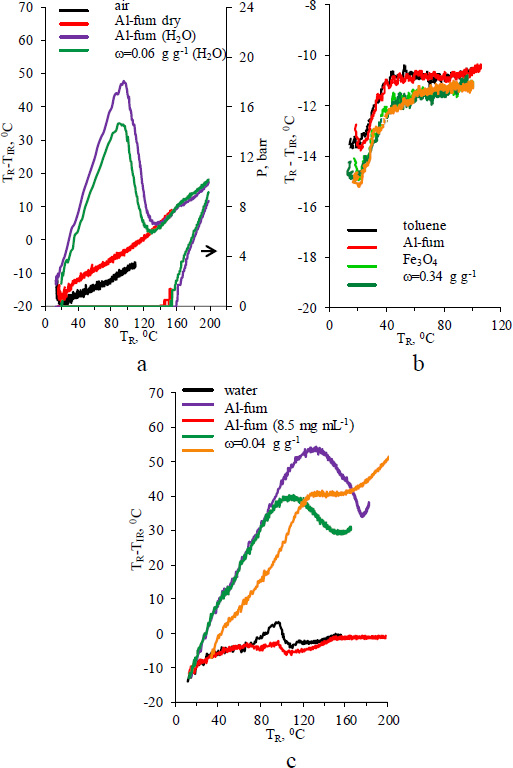
TR-TIR as a function of TR for Al-fum and Fe3O4/Al-fum composites: a) powder (corresponding pressure curves are shown in the left lower corner), b) toluene (8.5 mg mL-1), c) water (250 mg mL-1).
An analysis of temperature differences in spatially separated parts of a microwave reactor provides valuable information on the localization of materials that predominantly adsorb microwave radiation. The changes in temperature of the reactor content TR and its wall TIR and pressure P experimentally observed during microwave heating of dry and water-saturated MOFs are shown in Fig. (S1). For the vials filled with Al-fum and Fe3O4/Al-fum, the microwave heating curves are presented as TR-TIRvs. TR plots in Fig. (6). The dry powder of Al-fum seems to be reasonably transparent to a 2.45 GHz microwave radiation at ambient temperatures up to 120 0C Fig. (6a). Under these conditions, the vial wall temperature is higher than that of Al-fum powder (negative TR-TIR values). Therefore, the powder is warmed up by the heat supplied by the vial wall glass, which is apparently a more effective microwave-absorbing material in this case. A similar situation is typical for an air-filled vial. Gases, including water vapor, are poor absorbers of microwaves, and a difference between the readings of two thermometers is expected [41]. After the microwave heating for 18 min, the value of TR inside an air-filled vial asymptotically approaches 110 0C, being constantly 8 deg. lower than TIR.
After 4-5 min radiation of pre-dried and distilled toluene which is a low microwave-adsorbing solvent (tgδ=0.04) [41] TR of approximately 100 0C is reached (Fig. S2). Over a long period of time, the temperature difference remains equal to 11-12 0C in favor of the glass walls Fig. (6b). All dry Al-fum and magnetite/Al-fum composites do not change the TR-TIRvs. TR curve while dispersed in toluene in a concentration of 8.5 mg mL-1; the minor variations observed from sample to sample are within the experimental error.
The analysis of microwave absorption by dry Al-fum and composites in air and toluene suggests that in the absence of water, they are low microwave-absorbing materials below 100 0C, with their loss tangent apparently being comparable or lower than that of toluene. For dry Al-fum, the TR-TIR value slowly increases with increasing temperature and becomes positive above 120 0C Fig. (6a). This is presumably explained by changing dielectric permeability or loss of Al-fum at elevated temperatures. However, the available studies on dielectric properties of MOF cover so far only a few individual MOFs in limited frequency ranges [14-17, 42-44], among which Al-fum, to the extent of our knowledge, is not included. We can refer to the temperature dependence of dielectric properties for some known microwave radiation absorbers [45, 46] and another MOF at 1 MHz frequency [47].
Unlike dry Al-fum powder, the one saturated with water is heated extremely efficiently by microwaves (Fig. S1, c,d). TR rises to 120 0C in less than 2 min of heating. Moreover, the maximum temperature difference reaches 48 and 35 0C (while TR is equal to 90 and 95 0C) for Al-fum and a composite with ω= 0.06 g g-1 respectively Fig. (6a). The adsorption of microwave radiation of 2.45 GHz by a solvated Al-fum can be explained by electron delocalization induced by interactions between an adsorbate molecule and a MOF host. It leads to structural polarization, resulting in an enhancement of dielectric constant for the host−guest system as shown in the range of 1 Hz to 1 MHz for MFM-300(M) (M = Al, Sc, Cr, Fe, Ga, In) upon adsorption of Ar and NH3 [48], HKUST-1 upon loading with H2O, methanol, ethanol, and I2 [49-51], and UiO-66 upon adsorption of H2O [43].
The TR-TIR value decreases with further microwave heating, most likely due to the diffusion of heated water vapor from MOF to the walls. An almost linear increase of TR-TIR and pressure is observed over the range of elevated temperatures in which the MOF exhibits intrinsic microwave absorption.
Due to its medium value of loss tangent (tgδ=0.123 at 25 0C and 2.45 GHz), liquid water is a medium microwave-absorbing solvent [41]. Under experimental conditions, it is rapidly (within a 2 min period) heated up to ~110-120 0C, followed by a slow increase of temperature for 15 min to reach 160-190 0C (Fig. 7, S3). Because the dielectric loss and loss tangent of pure water decrease with increasing temperature [41], the absorption of microwave radiation by the solvent also decreases. Due to uniform heating and constant stirring of the liquid, the TR-TIR difference is minimal Fig. (6c). Similarly, in aqueous dispersions of Al-fum and composites with а concentration of 8.5 mg mL-1 under constant intensive stirring, the temperature gradient in the vial is negligible.
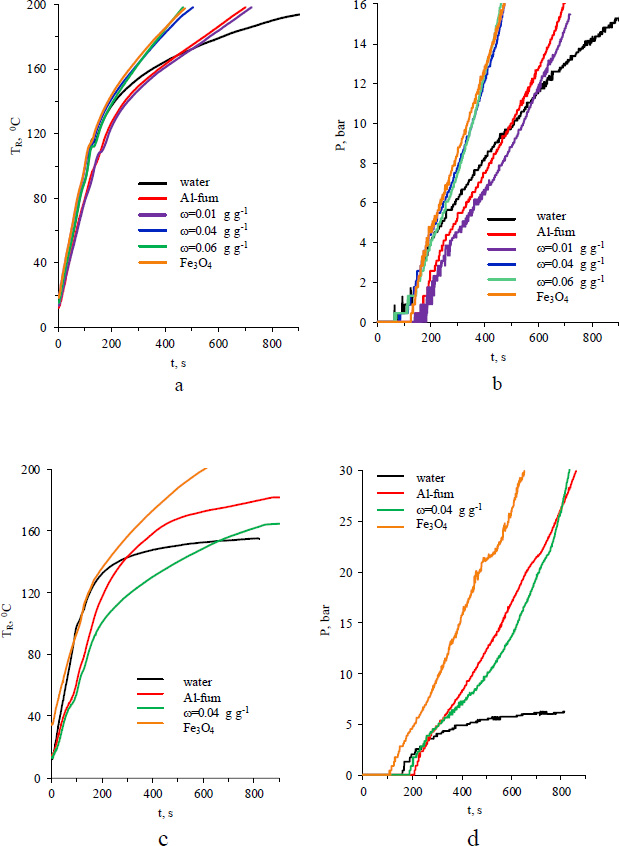
Temperature (a, c) and pressure (b, d) vs. time of microwave heating of Fe3O4/Al-fum composites at 8.5 (a, b) and 250 (c, d) mg mL-1.
As mentioned previously, water adsorbed inside MOF pores becomes an effective microwave absorber. A rapid increase in temperature inside the reactor is observed when aqueous dispersions of Al-fum or composite concentrated up to 250 mg mL-1 are irradiated (Fig. S4). The TR-TIR difference reaches the maximum values of 53 0С (at TR=128 0С) and 36 0С (at TR=106 0С) for Al-fum and Fe3O4/Al-fum dispersions accordingly Fig. (6c). The overheating of vial interior is apparently related to the low thermal conductivity (K) of aluminum fumarate due to its porous structure [52]. For Al-fum, the reported values of K vary from 0.1 to 0.3 W m−1 K−1, with a light dependence on dopant, temperature, coating thickness, and particle packing [53-55]. It is two times lower than the thermal conductivity of water.
In turn, magnetite has a much higher thermal conductivity as compared to water [56, 57] but also it is a highly effective microwave absorber in a wide range of microwave frequencies [57, 58].
Due to the increasing absorption capacity of the substance with increasing temperature, when treating substances with low thermal conductivity value, local overheating usually occurs and “hot spots” are formed because the released heat does not have time to be evenly distributed over the volume. For uniform heating of the substance by microwave radiation and elimination of uneven temperature distribution in the volume of the heated body, it is necessary that the substance has a sufficiently high thermal conductivity, such as magnetite [59, 60].
The positive TR-TIR values observed for the concentrated dispersion of Fe3O4 nanoparticles Fig. (6c) are most likely related to extreme heat production by magnetite particles under 2.45 GHz radiation and local overheating due to the absence of proper mixing.
Regardless of the investigated concentration, magnetite dispersions heat up faster than pure water Fig. (7). The fact is in good agreement with the microwave-related properties of magnetic nanoparticles [41]. For an Al-fum dispersion, the heating curve is located lower than that of water for temperatures below ~160-170 0C.
The effect of magnetite on microwave heating of Fe3O4/Al-fum composites depends on the mass fraction ω Fig. (8). At an 8.5 mg mL-1 concentration that ensures rapid redistribution of heat between parts of the reactor, only a composite with ω= 0.013 g g-1 shows the TR curve in the vicinity to that of unmodified Al-fum. The rest of the investigated Fe3O4/Al-fum composites demonstrate a rapid temperature increase, which is only a few degrees lower than that of pure magnetite dispersion. The magnetite mass fraction of 0.04 g g-1 is enough for accelerated heating of the dispersion, which is especially noticeable at temperatures above 160 0C. The time of microwave heating required to reach pre-set temperature of 200 0C decreases by 40-45% while using Al-fum or the composite with a low ω and by 60-65% if the mass fraction of magnetite is above 0.04 g g-1 (Fig. 8).
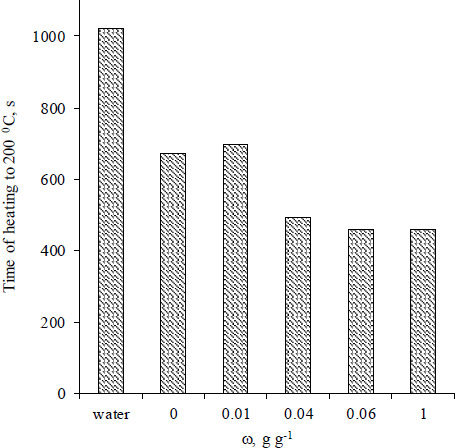
Time of heating to 200 0C for the Fe3O4/Al-fum composites with different ω.
In the Fe3O4/Al-fum composites obtained using pre-synthesized magnetite nanoparticles with a diameter of about 30 nm the magnetic phase is enclosed in the porous hydrophilic matrix of MOF. The mass fraction of magnetite in the composite varies from 0.01 to 0.34 g g-1 depending on the initial mass ratio of magnetite nanoparticles and aluminum salts in the reaction mixture. Both the synthesis of magnetic materials and metal-organic frameworks are implemented in industry. The proposed here synthetic approach to the magnetic MOF-based composites is believed to be fully scalable. The only scaling issue might be the preparation of magnetic particles of specified sizes from commercially available powders and long term stability of their aqueous dispersions, which imposes certain restrictions on the choice of magnetic phase for composites. Some aspects of this problem can apparently be solved by combining the stages of magnetic nanoparticle preparation and composite synthesis within one production facility.
It is shown that in such a composite magnetite completely retains its magnetic properties, but the Curie temperature decreases with decreasing the mass fraction of magnetic phase. A slight decrease in the Curie temperature by a maximum of 70 K occurs in this case, most likely due to the influence of hydrophilic Al fumarate matrix on the structure and properties of the surface layer of magnetite at the interface. It lies in the temperature range of more than 800 K, which is significantly higher than the temperature range of use of this composite - less than 500 K, which is limited by the temperature of its decomposition of aluminum fumarate (570 K). Therefore, this phenomenon will not affect the consumer properties of the material.
Moreover, it was shown that the temperature decrease usually occurs as a result of the formation of a thin layer (one nanometer) on the surface of particles that practically does not change their magnetization. For example, for a particle with a diameter of 30 nm, the volume of such a layer is only about 7% of the total volume of the particle. In the same limit, there is also a slight decrease in magnetization.
It was found that the measurement of the change in the Curie temperature of Fe3O4 in the composite is a sensitive marker indicating the interaction of the metal-organic framework matrix with the magnetic phase and can serve as a factor that will help in the future to characterize in detail the influence of MOFs on the magnetic properties of nanoparticles with which they form composites.
The broaden signals of the crystalline phase of magnetite in the X-ray spectra of the Fe3O4/Al-fum composites apparently can be associated with a large share of disordered magnetite crystal structure at the boundary of two phases. The mutual influence of two phases also appears as an increase in water uptake by a 0.04 g g-1 composite as compared with Al-fum matrix due to the escalation of microdefects in the MOF lattice structure caused by incorporated magnetite nanoparticles.
Among possible applications of magnetic MOF-based adsorbents, the magnetic separation must be mentioned first, with a drastically increasing rate of MOF removal after the adsorption of the target substance. Recently, an innovative proposal has been made to cool microchips using a MOF-based coating [61]. The use of the magnetite/Al-fum composites with enhanced and reproducible water vapor uptake and environmental stability will allow, in this case, to implement the thermal management of electronics through effects associated with the sorption/desorption of water vapor by MOF.
Conventional 2.45 GHz microwave equipment can be used to heat the Fe3O4/Al-fum composites while observing all standard safety precautions. No additional precautions are needed regardless of elevated pressures that are only encountered in a sealed container in the laboratory setting. However, if the magnetic MOF-based composites are intended to be used as a substitute for magnetite at significantly lower microwave frequencies, their ability to be heated up needs to be additionally investigated.
Dry Al-fum and the composites are poor microwave absorbers at temperatures below 120 0C, with slightly improved absorption at elevated temperatures. At the same time, due to the guest-host interaction between water molecules and the porous MOF matrix, the absorption of microwave radiation by Al-fum saturated with water is improved to a degree that allows one to overcome the decrease in dielectric losses and tangent of pure water with increasing temperature. As a result, water-enriched powders and aqueous dispersions of Al-fum and the Fe3O4/Al-fum composites are heated more evenly, almost linear in time, and allow for higher temperatures and pressures in a shorter period of time. The presence of magnetite in the composite in the amount of 0.04 g g-1 or more accelerates the heating of aqueous dispersions to 200 0C.
The use of induction heating with magnetite/MOF composite can have extensive practical applications in a large variety of processes where accelerated desorption is required. Magnetic nanoparticles, when exposed to a high-frequency magnetic field, heat up instantly, and when present within a MOF, this localized heating is enough to drive the release of adsorbed molecules.
There are three main mechanisms by which a substance transforms a microwave field into thermal energy. Dielectric heating refers to heating by the electric component of high-frequency electromagnetic radiation [59]. In addition to this, there are other types of microwave heating, such as magnetic loss heating, Joule heating caused by conductive losses, etc. Since MOFs have a fairly high resistance and are not magnetic, energy dissipation for them occurs only by the first mechanism [62].
Magnetite, being a magnetic material, is capable of releasing heat via all three mechanisms. It exhibits magnetic losses due to hysteresis, domain wall resonance, and, inherent to it, electron spin resonance - FMR (resonance mode of energy absorption) [63]. All these factors significantly increase the efficiency of heating the composite containing the magnetic phase since heat will be generated in this case under the influence of high-frequency electromagnetic radiation inside the material itself.
For example, in the process of oxygen separation from air using MOFs, owing to their thermally insulating nature and often favorable binding of guest species, controlled desorption of the adsorbed molecules from such frameworks can be challenging and energy intensive [64]. In this case, the use of the microwave heating effect of magnetic nanoparticles to achieve the desorption can improve the process.
CONCLUSION
Our finding on the effect of Fe3O4/Al-fum composites on the time of heating of aqueous suspensions allows them to be considered as additives which, while introduced into a given spatial area, can be heated locally to achieve a significant temperature difference, for example, in microwave-assisted 3D printing.
AUTHORS’ CONTRIBUTIONS
T. G. S.: Conceptualization, investigation, methodology, visualization, and writing – original draft, review and editing; A. S. T.: Investigation, formal analysis, and data curation; D. S. M.: investigation and formal analysis. K. S. L.: Data curation and writing – review & editing; V. V. P.: Conceptualization, data curation, and writing – review & editing. All authors have made a significant contribution to this work and have read and agreed to the published version of the manuscript.
LIST OF ABBREVIATIONS
XRD | = X-ray diffraction analysis |
RH | = Relative humidity |
P | = Pressure |
TIR | = Temperatures of the wall |
TR | = Internal content |
AVAILABILITY OF DATA AND MATERIALS
The data supporting the findings of the article will be available from the corresponding author [V.V.P.] upon reasonable request.
FUNDING
This work was supported by grants 8.1.3.5 and 8.1.3.6 from the State Program for Scientific Research “Materials Science, New Materials and Technologies” for 2021–2025, the Republic of Belarus.
ACKNOWLEDGEMENTS
Declared none.