All published articles of this journal are available on ScienceDirect.
Poly (Adenine) Modified Graphene-Based Voltammetric Sensor for the Electrochemical Determination of Catechol, Hydroquinone and Resorcinol
Abstract
Objective:
This paper presents the application of Poly (Adenine) Modified Graphene Paste Electrode (PAMGPE) for the analysis of Catechol (CC) with Resorcinol (RC) and Hydroquinone (HQ) by a voltammetric technique.
Methods:
Electropolymerization technique was utilized for the modification of the sensor surface. The electrode surface was characterized by Field Emission Scanning Electron Microscopy (FE-SEM). Cyclic Voltammetry (CV) and Differential Pulse Voltammetry (DPV) were used to study the redox behavior of CC, RC and HQ.
Results:
Oxidation peak current of CC increased linearly with the concentration of CC in the range from 2×10-6- 8×10-6 M and 1×10-5-1.5 ×10-4 M with a detection limit of 2.4×10-7 M. The practical application of the developed sensor was verified as exact for the determination of CC in water sample.
Conclusion:
The stability, repeatability, and reproducibility of the developed electrode were studied and established good characteristics. Furthermore, the PAMGPE was examined for the simultaneous determination of CC, RC and HQ.
1. INTRODUCTION
Sensitive and selective electrochemical techniques for the analysis of bioactive molecules with environmental and biological attention have been developed during the past few decades. The development of fast, sensitive, selective and correct electrochemical methods is very important for the field applications with various blocking contaminants. The voltammetric method is the best method for analyzing selective ion species [1-19].
Catechol (CC), resorcinol (RC), and hydroquinone (HQ) are widely used as industrial solvents. CC is widely used to produce food additive agents, hair dyes, and antioxidants [20]. RC is usually employed to produce dyes, plastics, and synthetic fibers [21-23]. Phenolic compounds, such as CC, RC, and HQ are found in the effluents of industries such as textile, paper and pulp, steel, petrochemical, petroleum refinery, rubber, dye, plastic, pharmaceutical, cosmetic, etc. and in the wastewater of synthetic coal fuel conversion processes [24, 25]. Catechol is also an important element necessary for metabolism in aromatic pollutants biodegradation [26]. Because catechol is soluble in water, it easily enters the habitat, which leads to its extensive spread in soil and water resources and thus causing a harm to nature and living organisms [27, 28]. Owing to its biotoxicity, catechol can cause lymphohematopoietic cancer, toxicity to the central nervous system, and DNA replication inhibition [29], and thus has been identified as a human carcinogen by the International Agency for Research on Cancer [30]. Therefore, the sensitive detection of catechol is of great significance for the treatment of catechol pollution and environmental monitoring. CC, HQ and RC are the kinds of dihydroxy benzene isomers extensively used as raw materials and synthetic intermediates in the chemical and pharmaceutical industries [31]. Attempts have been made to study the oxidation progress of HQ, RC and CC in electrochemical studies [32]. The rapid and accurate detection of these isomers in the aqueous environment is a prerequisite for their effective management and treatment, but in practice, it is difficult to distinguish HQ, RC and CC in environmental samples because of their similar chemical structure and properties [33]. In current years, many studies reported techniques for the detection of CC, such as chromatography [34], spectrophotometry [35] and fluorescence [36]. In addition continuous development and improvement of electrochemical sensors in combination with other modifiers, such as polymers, surfactants, CNT and ionic liquids [37-41] have been widely reported. Although electrochemical techniques have the advantages of accuracy and rapid determination of CC, RC and HQ compounds compared to other methods that require pretreatment before testing, their operation process is complicated; expensive instrument makes them not suitable for on-site detection, especially real-time detection. These disadvantages have limited the practical application of voltammetry to a certain extent. The method proposed in this study has attracted great attention because it is simple, sensitive, and low cost and can easily realize detection. To the best of our knowledge, detection of CC, RC and HQ using PAMGPE has not been investigated.
2. MATERIALS AND METHODS
2.1. Chemicals
Graphene was obtained from Tokyo Chemical Industries. CC and HQ were purchased from Sisco research laboratories, Mumbai, India. Silicone oil (binder) was obtained from Nice Chemical, India. Disodium hydrogen phosphate, monosodium dihydrogen phosphate and adenine were obtained from Himedia Chemicals Company, Bangalore, India. RC was obtained from Spectrum Reagents and Chemical Pvt Ltd. Cochin, India. A stock solution of CC (25×10-4 M), HQ (25×10-4 M) RC (25×10-4 M) and adenine (25×10-3 M) was prepared by dissolving in double-distilled water. PBS was prepared by mixing a suitable amount of 0.1 M disodium hydrogen phosphate and monosodium dihydrogen phosphate.
2.2. Apparatus
The electrochemical detection of CC, RC and HQ was carried out using a three-electrode system connected with CHI 6038E electrochemical workstation (USA). Saturated calomel electrode was used as a reference electrode, platinum electrode as a counter electrode, BGPE and PAMGPE as working electrodes. Before starting the measurements, approximately certain amount of paste from the carbon paste electrode was wiped off by a filter paper to obtain a new surface.
2.3. Electrode Preparation
Bare Graphene Paste Electrode (BGPE) was prepared by mixing 60% of graphene powder and 40% of silicone oil using a mortar and pestle. After homogenization, a portion of this mixture was firmly filled into the cavity of a Teflon tube and electrical contact was made by using a copper wire. For the construction of the polymer-modified electrodes, BGPE was electrochemically activated by using ten times cyclic potential sweeps in the range from 0 to 1.6 V in 0.1 M PBS (pH 6.5) at a scan rate of 0.1 Vs−1 with 0.1 mM adenine (Fig. 1). After electropolymerization, the modified electrode was rinsed with distilled water.
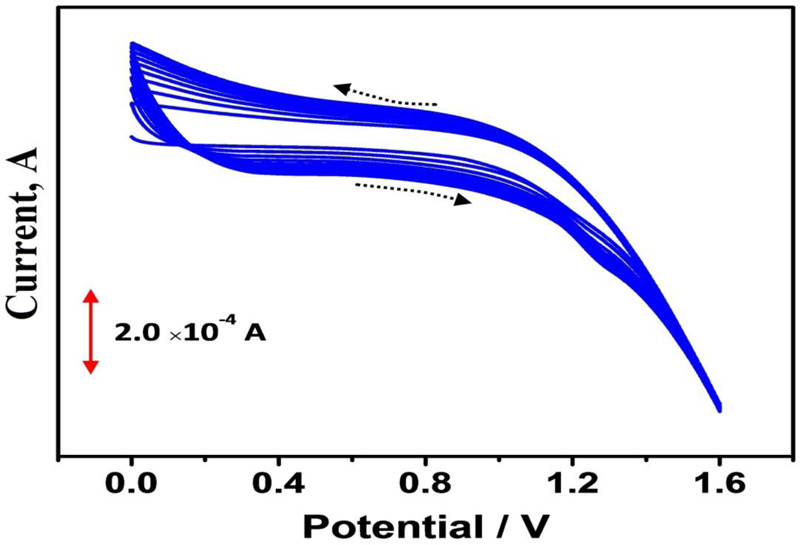
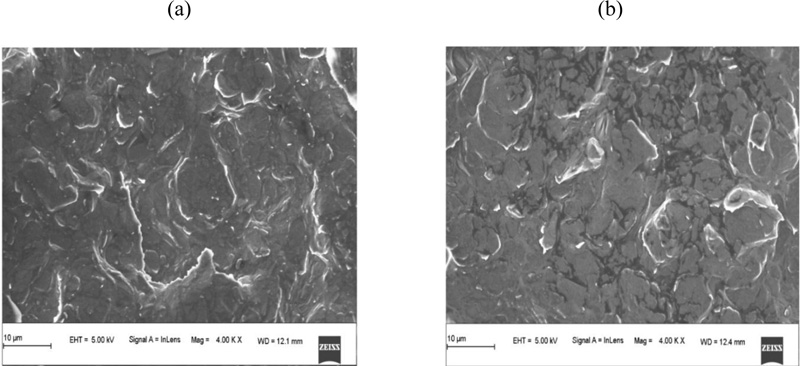
3. RESULTS AND DISCUSSION
3.1. Morphological Characterization of BGPE and PAMGPE
Fig. (2) shows the FESEM images of the BGPE and PAMGPE. The surface of the BGPE showed irregularly shaped graphene flakes and separated layers (Fig. 2a). The FESEM image of PAMGPE (Fig. 2b) shows the formation of a polymer layer on the BGPE surface. PAMGPE not only enlarged the surface area but also improved the electron transfer rate.
3.2. Electrochemical Response of CC at PAMGPE
The electrochemical response of CC was studied by performing CV at BGPE and PAMGPE in a solution containing 1×10−4 M CC in 0.1 M PBS (pH 7) with the scan rate of 0.1 V/s Fig. (3). The CV plots of CC at BGPE (solid line) show an anodic peak current at 5.64 μA and cathodic peak current at 4.9 μA. The ratio of the peak current indicates the quasi-reversible oxidation of CC at the electrode surface. It was also observed that the electrochemical response of CC at PAMGPE (dotted line) is considerably enhanced in terms of both peak current and peak shape (anodic peak current at 111.2 μA, and cathodic peak at 96.9 μA) compared to BGPE. This implies that the oxidation of CC is more favorable on the surface of PAMGPE than BGPE.
3.3. Electrochemical behaviors of CC by DPV
Fig. (4) shows DPVs of 1×10−4 M CC in 0.1 M PBS (pH 7.0) at BGPE (solid line) and PAMGPE (dotted line) with the potential scan rate of 0.05 V/s. Peak current obtained at PAMGPE was much higher than the peak current obtained at BGPE. High surface area and conductivity of polymer film played an important role, which made the transfer of electrons easier.
3.4. Electrocatalytic Oxidative Reaction of CC
The voltammetric behavior of CC (1×10-4 M) in the presence (dotted line) and absence (solid line) at PAMGPE is displayed in Fig. (5). The cyclic voltammograms clarify that there was no anodic peak and cathodic peak response in the case of blank, but in the presence of CC, a good voltammetric peak response was observed with the anodic peak current of 111.2 µA and peak potential of 0.155 V. This data shows that the electrocatalytic oxidative reaction depends only on CC at PAMGPE.
3.5. Effect of Scan Rate on the Peak Current
The variation in the scan rate at the peak current of 1 × 10−4 M CC in 0.1 M PBS (pH 7.0) was examined in the range from 0.1–0.3 Vs–1 (Fig. 6a). The plot of the peak currents (Ip) against the scan rate (v) exhibited a linear relationship from 0.1- 0.3 V/s with a regression equation of Ipa (μA) = 715.4v (mVs−1) – 47.26 (r2 = 0.997) (Fig. 6B). This specifies that the electrode reaction is an adsorption controlled process. When the scan rate increased, the peak potential slightly shifted to positive values. The linear relationship between the peak potential and the logarithm of the scan rate was obtained to be Ep(V) = 0.2297 log v (V s−1) + 0.1925; r2 = 0.997 (Fig. 6c). This specifies that the electrode reaction is an adsorption controlled process.
3.6. The Effect of Solution pH
The effect of solution pH on the electrocatalytic oxidation of CC at PAMGPE was studied by the CV technique in the pH range from 6.0–8.0 (Fig. 7a). With an increase in the solution pH, anodic peak potentials shifted to negative values. This specifies that the electrocatalytic oxidation of CC at PAMGPE is a pH-dependent reaction and shows that protons were involved in the electrode reaction [14]. The highest oxidation peak current was obtained at pH 7 (Fig. 7b), after that, it decreased. Epa was proportional to the pH over the range from 6-8 with the linear regression equation of Epa (mV) = 585-56.4 pH [37], with a correlation coefficient of r2= 0.921 (Fig. 7c).
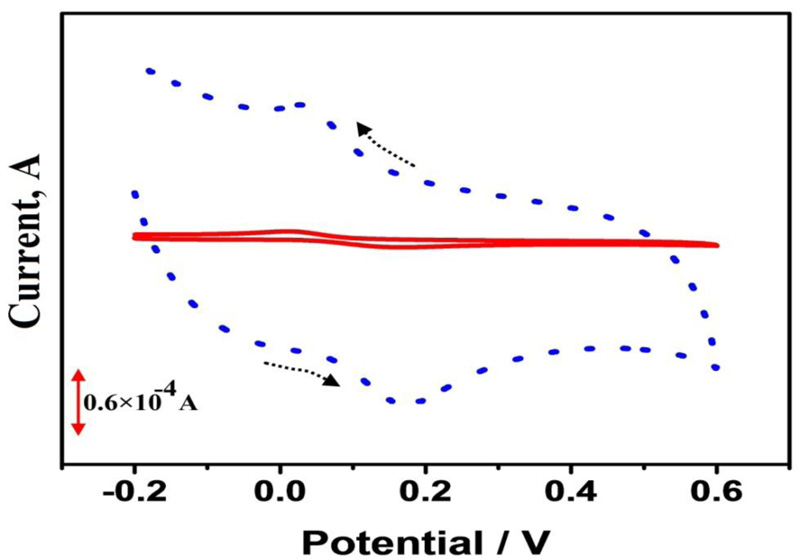
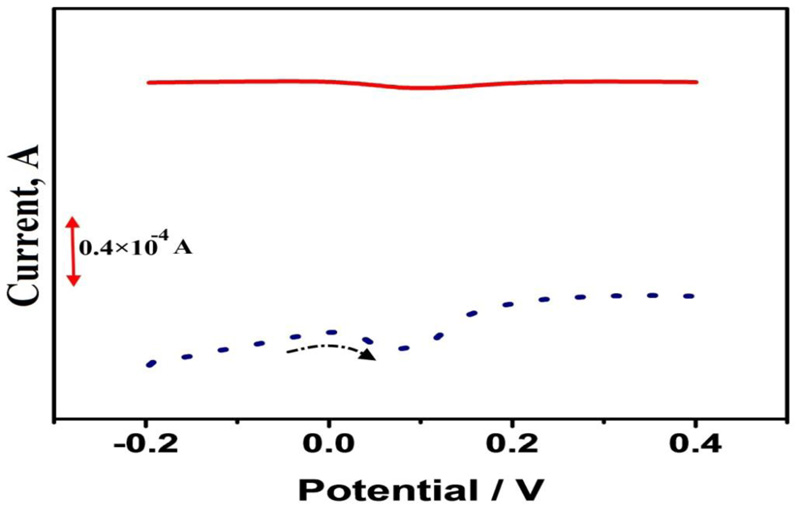
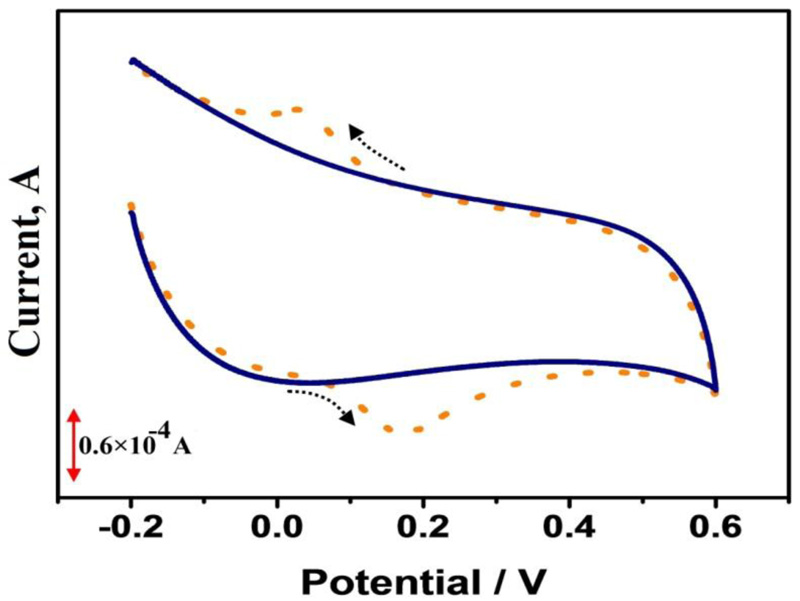
3.7. Repeatability, Stability, and Reproducibility
The repeatability of the developed sensor towards CC (1×10-4 M) was investigated by using three continuous measurements with the same electrode, resulting in RSDs of 2.5%. This indicates the good repeatability of the developed sensor. The storage stability of the sensor was examined by intermittently measuring the peak current response of 1×10-4 M CC every ten days over one month (stored in an airtight environment at room temperature) and the response declined by 9.79% from its original response after the 30-day storage, which specifies that the modified electrode has good stability. The reproducibility sensor was evaluated by measuring the current response of three sensors constructed independently under identical experimental conditions, and the RSD was 2.65%. This shows the good reproducibility of the developed sensor.
3.8. Responses of HQ at PAMGPE
As shown in Fig. (8a), the sensing response of HQ at PAMGPE (ΔI = 7.6μA) is ~ 3 times higher than that observed at BGPE (ΔI = 2.6μA) and the ratio of peak current indicates the quasi-reversible oxidation of HQ at the electrode surface. Fig. (8b) shows the effect of different scan rates on HQ oxidation at PAMGPE by using CV. With the increase in the scan rate from 0.1 to 0.250 V s−1, the anodic peak current also increased linearly with the linear regression equation of Ipa (A) = 1.975 + 0.0584 ν (V s−1) and a correlation coefficient of 0.998 (Fig. 8c). This specifies that the electrochemical reaction is consistent with the adsorption-controlled process.
3.9. Electrochemical Behavior of RC at PAMGPE
Fig. (9a) shows the cyclic voltammogram of 1×10-4 M of RC at BGPE (dotted line) and PAMGPE (solid line) in 0.1 M PBS (pH 7) at the scan rate of 0.1 V/s. RC shows a weak response at BGPE, which is 1.73 times higher than that at PAMGPE . From the graph, it is clear that the oxidation of RC is irreversible. The enhancement in Ipa at PAMGPE compared with BGPE may be due to the high electron transfer kinetics, high surface area and good conductivity, which accelerate electron transfer processes between RC and PAMGPE. The influence of the potential scan rate on electrocatalytic oxidation of RC was studied by CV. As seen in Fig. (9b), with the increasing scan rate from 0.1 to 0.2 Vs−1, the oxidation peak potential shifts to a more positive potential, showing the kinetic limitation in the electrochemical reaction. A linear relationship between Ip and υ (Fig. 9c) was observed with the linear regression equation of Ipa (A) = 7.096 + 0.037ν (V s−1) and a correlation coefficient of 0.993, which demonstrates that the electrode process is controlled by the adsorption.
3.10. Simultaneous Determination of CC, RC, and HQ
The principal target of this study was to develop a method for the simultaneous separation of CC, RC, and HQ using PAMGPE. Under the optimized conditions, the simultaneous determination of CC, RC, and HQ was studied using CV (Fig. 10). Three well-separated anodic peaks were considered at potentials of 0.044, 0.179 and 0.543 V, which corresponded to the oxidation of HQ, CC, and RC. The potential difference between the three anodic peak potentials of HQ, CC, and RC were 0.13, 0.36 and 0.49 V, respectively, was high enough for the simultaneous determination of the concentration of CC, RC, and HQ. All three molecules’ oxidation mechanisms are proposed in Scheme. (S1).
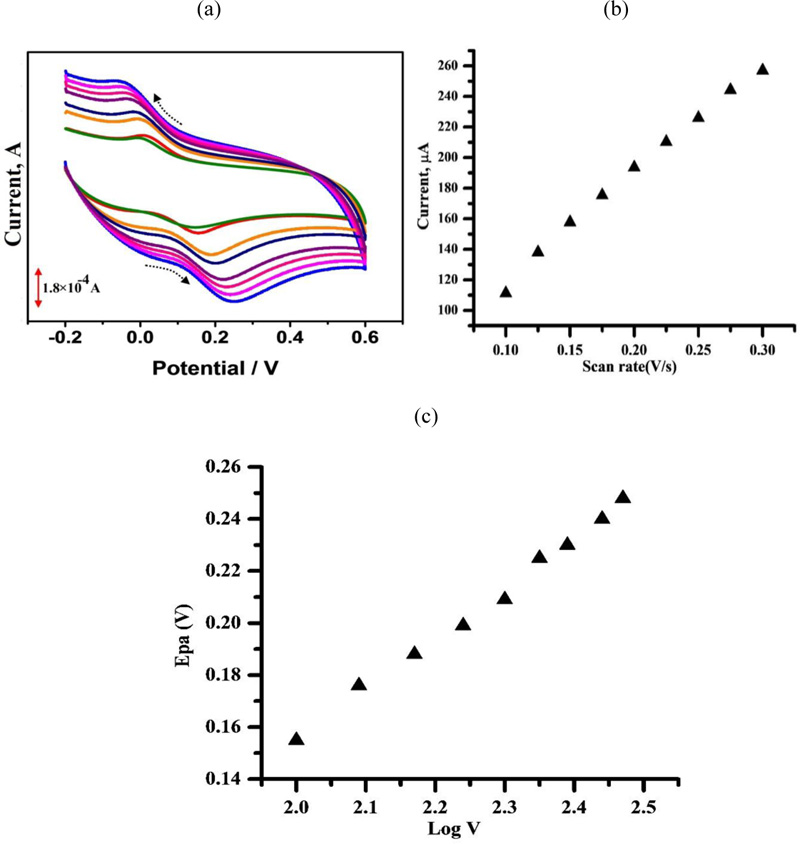
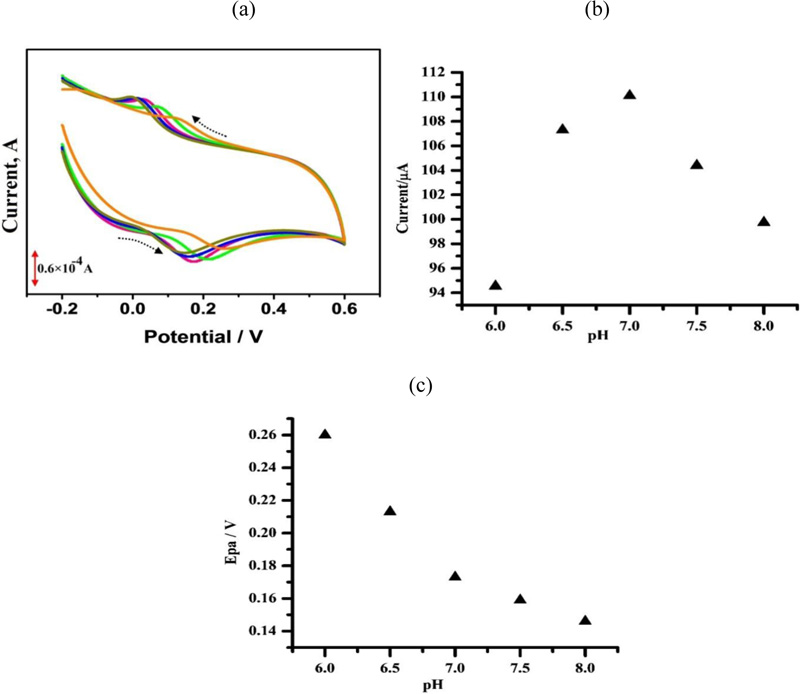
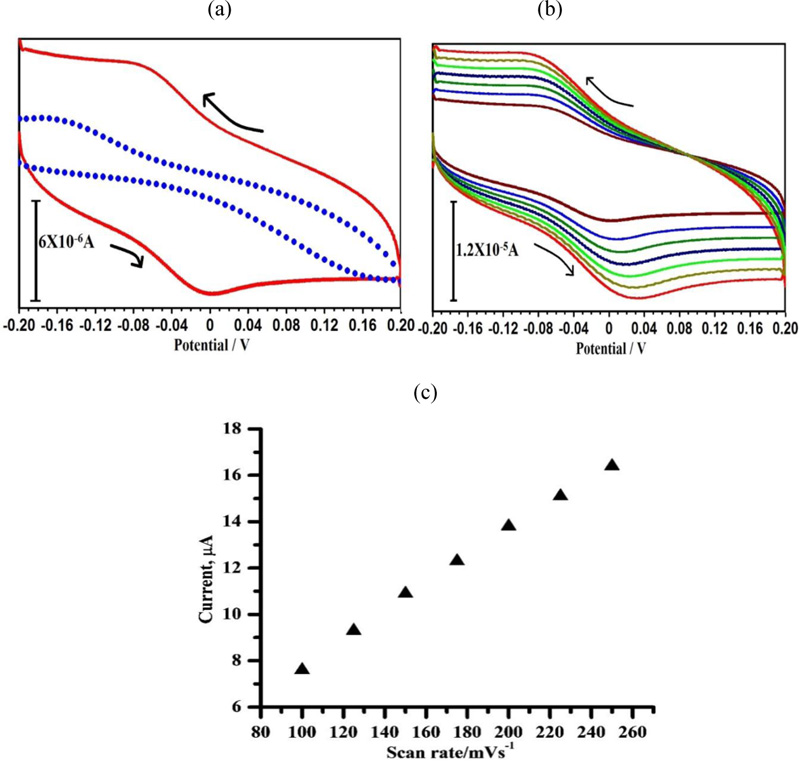
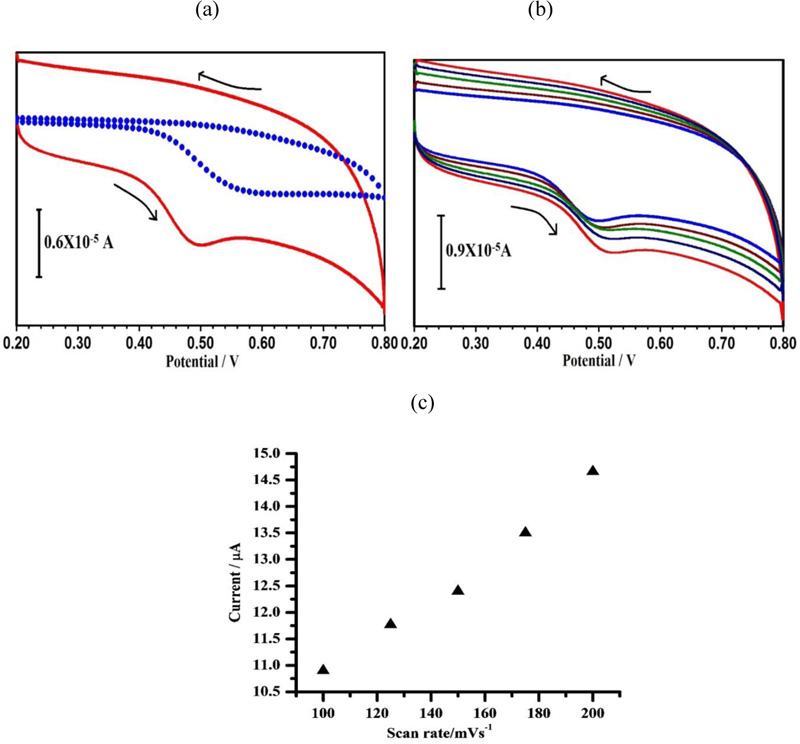
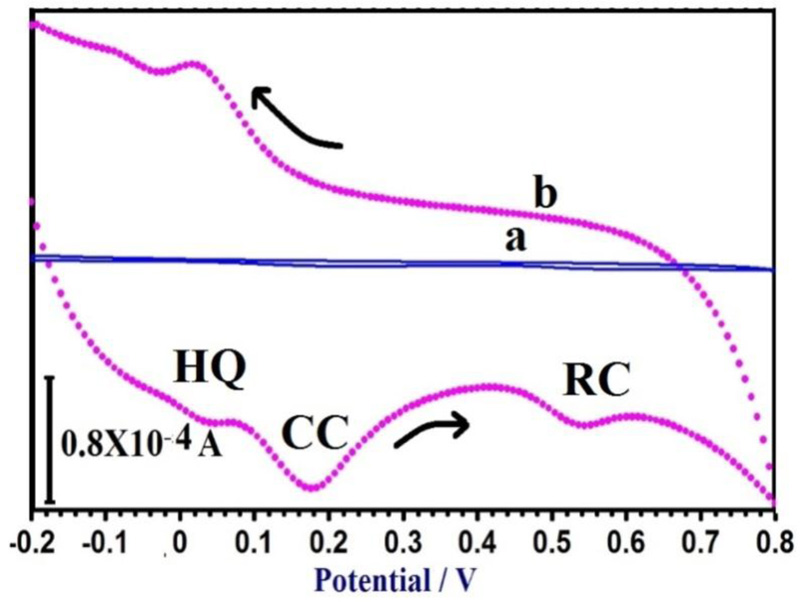
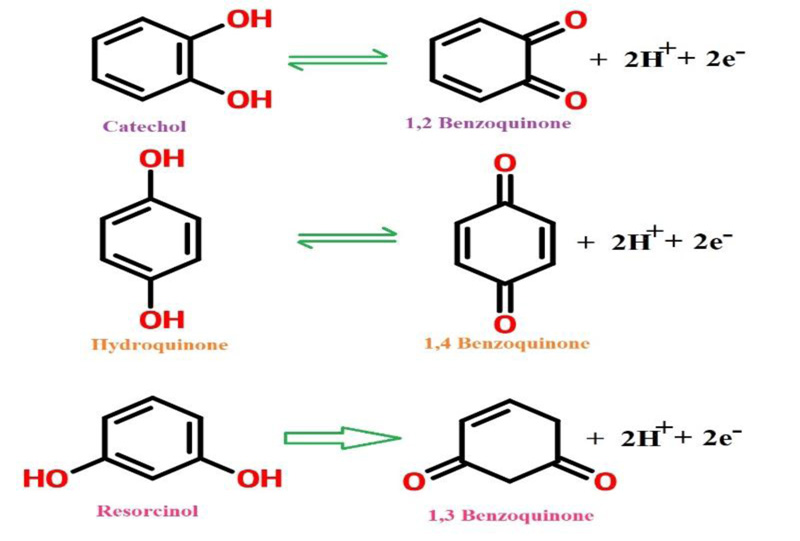
3.11. Influence of CC Concentration
Graphene paste electrode modified with poly (adenine) indicated a significant increase in voltammetric sensitivity. CV was conducted by successively adding aliquots to the electrochemical cell. The analytical curve was obtained by the two linearities over the concentration range from 2×10-6- 8 ×10-6 M and 1×10-5- 1.5 ×10-4 M. The correlation coefficient (r) was 0.993 and 0.991 with a (Fig. 11) standard deviation (SD) of 5.303× 10-7 and 1.726×10-7, respectively. The linear regression equation was obtained to be; Ipa (A) = 8.10×10-5+1.460 C (M). Using the relation 3 SD/m and 10 SD/m (where, SD is the standard deviation of the blank solution and m is the voltammetric sensitivity of the curve), the obtained values of limit of detection (LOD) and limit of quantification (LOQ) were 2.4×10-7 M and 9.1×10-7 M. Comparisons of the results from the different published paper methods are shown in Table 1 [42-50].
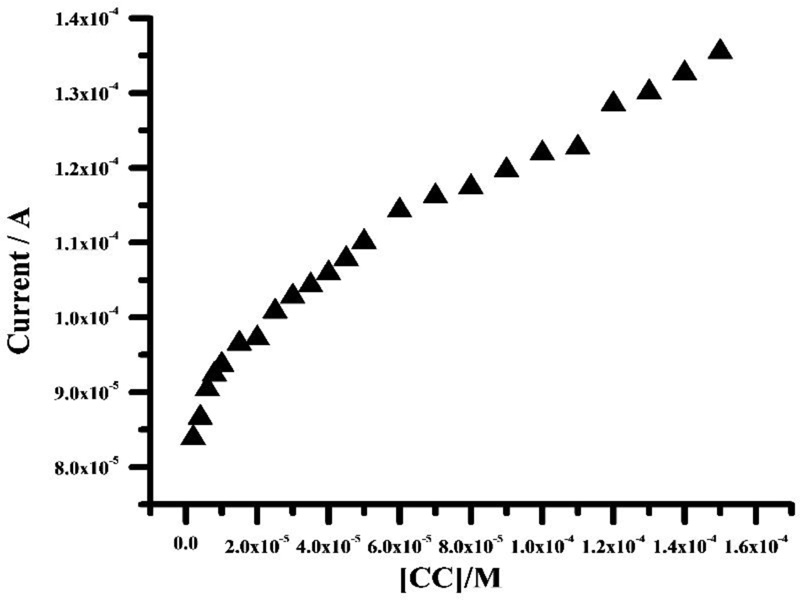
Working Electrode | Limit of Detection | Method | References |
---|---|---|---|
In µM | |||
RGO–MWNTs | 1.8 | DPV | [42] |
MWCNT–NF–PMG/GCE | 31 | CV | [43] |
Influence of micelles/GCE | 3 | DPV | [44] |
CNx/GCE | 2.71 | LSV | [45] |
[Cu(Sal-β-Ala)(3,5- | 3.5 | DPV | [46] |
DMPz)2]/MWCNTs/GCE | |||
LDHf/GCE | 9 | DPV | [47] |
Poly(glycine) MGPE | 0.87 | CV | [48] |
Poly(methionine) MCPE | 55.66 | CV | [49] |
Eosin Y/CPE | 0.27 | CV | [50] |
Poly (adenine) MGPE | 0.24 | CV | This work |
3.12. Analytical Applications
To prove the applicability of the modified electrode for the determination of CC, PAMGPE was applied for the determination of CC in the water sample. The CC measurement was performed by applying the proposed modified electrode in real sample solution at pH 7 using CV. The recoveries were obtained in the range from 92.04 to 98.5%, revealing that the modified electrode was very effective for the determination of CC in the water sample with a good recovery rate.
CONCLUSION
In this work, PAMGPE was developed for the determination of CC, RC and HQ. Peak current enhancement and reduced peak potential were observed at the modified electrode. A brief comparison of this work with other electroanalytical methods was reported. The proposed PAMGPE has many advantages such as easy preparation, real-time sample analysis without pretreatment, good stability, and enhanced selectivity. Moreover, in comparison with the other procedures, the PAMGPE indicated good results in terms of selectivity, sensitivity and the detection limit.
CONSENT FOR PUBLICATION
Not applicable.
AVAILABILITY OF DATA AND MATERIALS
Not applicable.
FUNDING
None.
CONFLICT OF INTEREST
The authors declare no conflict of interest, financial or otherwise.
ACKNOWLEDGEMENTS
Declared none.